|
|
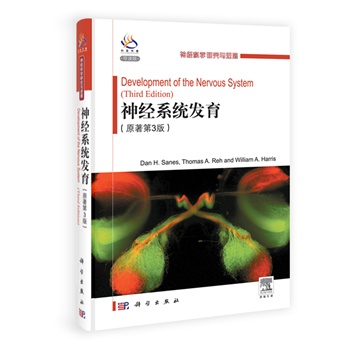 |
《神经系统发育(原著第三版)(导读版)》 |
作者:谢治慧、景乃禾 |
出版社:科学出版社 |
出版日期:2012/1/1 |
ISBN:9787030329073 |
定价: 118.00元 |
|
内容推荐
《神经系统发育》(第3版)由三位知名学者主笔,以现在和既往的重要实验与观察结果为例,对业已建立的和正在演变的神经发育原理进行广泛和基础的讨论。
《神经系统发育(原著第3版)》按照个体发生的顺序组织内容。从出现神经原基开始,随后每一章节按神经发育事件出现的顺序组织:神经系统的模式建成和生长,神经元命运决定,轴突导向和靶点寻找,神经元存活与死亡,突触形成与发育的可塑性。在结构部分基本完成后,最后一章讨论了行为的出现。
新版的《神经系统发育》反映了通过新的分子遗传学和细胞生物学方法的应用取得的最新成果。丰富的彩色照片和原始绘图,辅以简明的叙述,使《神经系统发育(原著第3版)》非常适合这一有趣领域的初涉者,包括高年级本科生、研究生和研究人员。
作者简介
Dan H.Sanes教授,纽约大学神经科学中心Thomas A.Reh教授,华盛顿大学生物结构系William A.Harris教授,剑桥大学生理、发育及神经科学系
目录
第三版序
第二版序
第一版序
1.神经诱导
神经元的发育与进化
多细胞动物的早期胚胎学
神经组织的衍化
神经组织产生时与相邻组织的相互作用
神经诱导物的分子属性
神经诱导的保守性
调控神经母细胞分离时外胚层细胞间的相互作用
小结
参考文献
2.极性与分节
神经系统的区域性特征
前-后轴与hox基因
Hox基因在脊椎动物神经系统中的功能
调控脊椎动物前-后轴模式建立的信号分子:头或尾
脑发育的组织中心
前脑发育,前脑结(prosomeres)与pax基因
神经管的背-腹极性
背侧神经管与神经脊
大脑皮层模式的建立
小结
参考文献
3.发生与迁移
什么决定由前体细胞产生的细胞数目?
神经元与胶质细胞的产生
大脑皮层的组织发生
小脑皮层的组织发生
神经元迁移的分子机制
胚胎后期与成体的神经发生
小结
参考文献
4.命运决定与分化
细胞谱系的转录调控组织次序:线虫神经元
命运决定过程的时间与空间协同:果蝇中枢神经系统的神经母细胞
不对称细胞分裂与细胞命运决定
细胞间相互作用产生复杂性:果蝇视网膜
细胞间相互作用和与微环境的相互作用决定细胞命运:脊椎动物神经脊细胞
响应性与组织发生:哺乳动物大脑皮层
组织发生过程中内外因素的整合:脊椎动物视网膜
形成素的浓度梯度与细胞类型的空间组织:脊髓运动神经元
小结
参考文献
5.轴突生长与导向
生长锥
动态的细胞骨架
树突的形成
生长锥怎样持续生长?
什么给生长锥提供导向信号?
细胞黏附及示踪的通路
排斥性导向信号
趋化性,梯度与局部信号
信号转导
中线:穿过还是不穿过?
吸引与排斥:脱敏及适应性
视觉通路:从这里到那里
小结
参考文献
6.靶点区选择
轴突解聚
靶点区识别与进入
在靶点区减速并形成分支
边界巡防:阻止不当的靶向选择
定位图绘制
化学专一性和ephrins
三维的、板层特异的终末定位
细胞和突触的靶向
发现靶点区
移动与连接的精细调控
小结
参考文献
7.自然发生的神经元死亡
神经元死亡时呈现的形态
早期淘汰的前体细胞
有多少已分化的神经元死亡?
神经元存活依赖于突触靶点区的选择
神经营养因子(NGF):靶点区产生的促存活因子
神经营养素家族
神经营养素受体:酪氨酸受体激酶(TRK)家族
神经营养蛋白信号是如何到达细胞体的?
p75神经营养素受体能启动细胞死亡过程
细胞因子作为促神经元存活因子
激素控制的神经元存活
神经元死亡需要蛋白合成
介导神经元存活的细胞内信号通路
介导神经元死亡的细胞内信号通路
半胱氨酸天冬氨酸蛋白酶(Caspases):死亡因子
Bcl-2蛋白:细胞程序性死亡的调控因子
清除正在死亡的神经元
靶点区的突触传递
神经传入调节神经元存活
细胞内钙信号同时介导神经元存活与死亡
小结
参考文献
8.突触形成与功能
新形成的突触是什么样的?
突触在突触后细胞的哪个位置形成?
新形成的突触要多久才能整合到神经系统
突触形成的最初标志
决定形成突触
黏性突触
生长锥转变为突触前终板
神经肌肉接头(NMJ)的受体聚合与突触后分化
Agrin是存在于NMJ的跨突触聚合信号分子
中枢神经系统的受体聚合信号
中枢神经系统的支架蛋白(Scaffold protein)与受体聚集
神经支配增加受体表达和嵌入
突触活性调节受体密度
信号传递的成熟与受体亚型的转变
递质重摄取过程的成熟
短期可塑性
突触抑制的出现
在发育过程中突触抑制是否真是抑制性的
小结
参考文献
9.突触连接的精细化
突触连接的早期模式
功能性突触的清除
许多轴突分支被清除或被精细化
感觉环境影响突触联系
神经活动影响NMJ突触的清除
感觉信息编码特性反映突触的精细化
神经活动有助于定位图谱的形成和重排
自发电活动和神经传入的精细化
关键时期:发育中突触可塑性的强化
异源突触抑制和突触的清除
胞内钙信号的参与
钙信号激活的第二信使系统
增益效应
稳态可塑性:改变越多,越能保持原状
抑制性突触连接的可塑性
突触对神经元形态的影响
小结
参考文献
10.行为发育
行为的个体发生
最初的运动是自发的
自发运动的机制
更复杂的行为是由简单的神经环路整合装配而成
神经活动在协调性行为出现中的作用
阶段特异性行为
行为的遗传决定因素
行为发育的环境决定因素
开始感知世界
对婴儿提问(并得到一些应答!)
敏锐的听觉
锐利的视觉
性别特异的行为
遗传性别
激素调控脑的性别
在脑中唱歌
遗传控制果蝇的脑性别
从基因组到脊椎动物的脑性别
基因组印记:亲代控制的根本原则
立即开始学习
由厌恶产生的学习偏好
技能学习:它并不容易获得
从脑与脑之间获得信息
语言
小结
参考文献
分子与基因名称索引
专业名词索引
在线试读部分章节
Chapter 1 Outline
Development and evolution of neurons 1 Early embryology of metazoans 1 Derivation of neural tissue 2 Interactions with neighboring tissues in making neural tissue 7 The molecular nature of the neural inducer 10 Conservation of neural induction 13 Interactions among the ectodermal cells in controlling neuroblast
segregation 17 Summary 21 References 21
DEVELOPMENT AND EVOLUTION OF NEURONS
Almost as early as multicellular animals evolved, neurons have been part of their tissues. Metazoan nervous systems range in complexity from the simple nerve net of the jellyfish to the billions of specifically interconnected neuron assemblies of the human brain. Nevertheless, the neurons and nervous sys-tems of all multicellular animals share many common features. Voltage-gated ion channels are responsible for action poten-tials in the neurons of Jellyfish as they are in people. Synaptic transmission between neurons in nerve nets is basically the same as that in the cerebral cortex in humans (Figure 1.1). This book describes the mechanisms responsible for the gener-ation of these nervous systems, highlighting examples from a variety of organisms. Despite the great diversity in the nervous systems of various organisms, underlying principles of neural development have been maintained throughout evolution.
It is appropriate to begin a book concerned with the devel-opment of the nervous system with an evolutionary perspec-tive. The subjects of embryology and evolution have long shared an interrelated intellectual history. One of the major currents of late-nineteenth-century biology was that a descrip-tion of the stages of development would provide the key to the path of evolution of life. The phrase “ontogeny recapitu-lates phylogeny” was important at the start of experimental embryology ( Gould, 1970 ). Although the careful study of embryos showed that they did not resemble the adult forms of their ancestors, it is clear that new forms are built upon the
. 2012 Elsevier Inc. All rights reserved.
structures of biological predecessors. One aim of this book is to show how an understanding of the development of the ner-vous system will give us insight into its evolution. It is also wise to remember, as Dobzhansky pointed out, “nothing in biology makes sense except in the light of evolution.”
EARLY EMBRYOLOGY OF METAZOANS
The development of multicellular organisms varies substan-tially across phyla; nevertheless, there are some common fea-tures. The cells of all metazoans are organized as layers. These layers give rise to the various organs and tissues, including the nervous system. These layers are generated from the egg cell through a series of cell divisions and their subsequent rear-rangements. The eggs of animals are typically polarized, with differences in their cytoplasm from one “pole” to the other. Amphibians, for example, have an “animal pole” and a “vegetal pole” that is visible in the egg, since the vegetal pole contains the yolk, the stored nutrient material necessary for sustaining the embryo as it develops. Once fertilized by the sperm, the egg cell undergoes a series of rapid cell divisions, known as cleavages. There are many variations of cleavage patterns in embryos, but the end result is that a large collection of cells, the blastula, is generated over a relatively short period of time. In many organisms the cells of the blastula are arranged as a hollow ball, with an inner cavity known as a blastocoel. The rearrangement of this collection of cells into the primary (or germ) layers is called gastrulation. Gastrulation can occur via a variety of mechanisms, but all result in an inner, or endo-dermal, layer of cells, an outer layer of cells, the ectoderm, and a layer of cells between the two other layers, known as the mesoderm ( Gilbert and Raunio, 1997 ). The middle layer can be derived from either the ectoderm (ectomesoderm) or the inner layer (endomesoderm). During the process of gas-trulation, the cells of the mesoderm and endoderm move into the inside of the embryo, often at a single region, known as the blastopore. Once the endoderm and mesoderm are inside the ball, they usually obliterate the blastocoel and form a new cav-ity, the archenteron, or primitive gut. Animals can be divided in two on the basis of whether the mouth forms near the point of this blastopore (in protostomes) or at a distant site (in deu-terostomes). Once these three primary germ layers are estab-lished, the development of the nervous system begins. A more detailed description of the development of the other organ sys-tems is beyond the scope of this text. Nevertheless, one should keep in mind that the development of the nervous system does 1
DEVELOPMENT OF THE NERVOUS SYSTEM
Protists
Colonial flagelates
Radiata
Sponges
Bilateria Cnidarians
Deuterostomes Protostomes
Nematodes Platyhelminthes
Echinoderms Molluscs
Annelids
Tunicates
Vertebrates Arthropods
Fig. 1.1 Neurons throughout the evolution of multicellular organisms have had many features in common. All animals other than colonial flagellates and sponges have recognizable neurons that are electrically excitable and have long processes. The Cnidarians have nerve networks with electrical synapses, but synaptic transmission between neurons is also very ancient.
not take place in a vacuum, but is an integral and highly integrated part of the development of the animal as a whole.
The next three sections will deal with the embryology of several examples of metazoan development: nematode worms (Caenorhabditis elegans); insects (Drosophila melanogaster); and several vertebrates (frogs, fish, birds, and mammals). The development of these animals is described because they have been particularly well studied for historical and practical reasons. However, one should take these examples as repre-sentative, not as definitive. The necessity of studying many diverse species has become critical to the understanding of the development of any one species.
DERIVATION OF NEURAL TISSUE
The development of the nervous system begins with the segregation of neural and glial cells from other types of tissues. The many differences in gene expression between neurons 2 and muscle tissue, for example, arise through the progressive
narrowing of the potential fates available to a blast cell during development. The divergence of neural and glial cells from other tissues can occur in many different ways and at many different points in the development of an organism. However, the cellular and molecular mechanisms that are responsible for the divergence of the neural and glial lineages from other tissues are remarkably conserved.
C. ELEGANS
The development of C. elegans, a nematode worm, high-lights the shared lineage of the epidermal and neural cell fates. These animals have been studied primarily because of their simple structure (containing only about a thousand cells), their rapid generation time (allowing for rapid screen-ing of new genetic mutants), and their transparency (enabling lineage relationships of the cells to be established). These nematodes have a rigid cuticle that is made of collagenous proteins secreted by the underlying cells of the hypodermis. The hypodermis is analogous to the epidermis of other ani-mals, except that it is composed of a syncytium of nuclei rather than of individual cells. They have a simple nervous system, composed of only 302 neurons and 56 glial cells. These neurons are organized into nerve cords. The nerve cords are primarily in the dorsal and ventral sides of the ani-mals, but there are some neurons that run along the lateral sides of the animal as well. The nematodes move by a series of longitudinal muscles, and they have a simple digestive system.
Nematodes have long been a subject for developmental biol-ogists’ attention. Theodore Boveri studied nematode embry-ology and first described the highly reproducible pattern of cell divisions in these animals in the late 1800s. Boveri’s most famous student, Hans Spemann, whose work on amphibian neural induction will be described below, worked on nema-todes for his Ph.D. research. The modern interest in nematodes, however, was motivated by Sydney Brenner, a molecular biol-ogist who was searching for an animal that would allow the techniques of molecular genetics to be applied to the develop-ment of metazoans ( Brenner, 1974 ).
Because of the stereotypy in the pattern of cell divisions, the lineage relationships of all the cells of C. elegans have been determined ( Sulston et al., 1983 ). The first cleavage produces a large somatic cell, the AB blastomere, which gives rise to most of the hypodermis and the nervous sys-tem and the smaller germline P cell, which in addition to the gonads will also generate the gut and most of the muscles of the animal (Figure 1.2 ). Subsequent cleavages produce the germ cell precursor, P4, and the precursor’s cells for the rest of the animal: the MS, E, C, and D blastomeres ( Figure 1.2 ), and these cells all migrate into the interior of the embryo, while the AB-derived cells spread out over the outside of the embryo completing gastrulation (Figure 1.3). The next phase of development is characterized by many cell divisions and is known as the proliferation phase. Then an indentation forms at the ventral side of the animal marking the beginning of the morphogenesis stage, and as this indentation progresses, the worm begins to take shape ( Figure 1.3 ). At this point, the worm has only 556 cells and will add the remaining cells (to the total of 959) over the four larval molts. The entire development of the animal takes about two days.
1. NEURAL INDUCTION
time
P0
AB P1
LR LR
ABa ABp
EMS P2
MS C P3
D P4
Hypodermis and Body, muscles, gut, nervous system somatic gonad Germ line
Fig. 1.2 The nervous system shares a common cellular lineage with the ectoderm. The cell divisions that generate the C. elegans nematode worm are highly reproducible from animal to animal. The first division produces the AB blastomere and the P1 blastomere. The germ line is segregated into the P4 blastomere within a few divisions after fertilization. The subsequent divisions of the AB blastomere go on to give rise to most of the neurons of the animal, as well as to the cells that produce the hypodermis―the epider-mis of the animal.
The neurons of C. elegans arise primarily from the AB blastomere, in lineages shared with the ectodermally derived hypodermis. An example of one of these lineages is shown in Figure 1.3 . The Abarpa blastomere can be readily identified in the 100-min embryo through its position and lineal history. This cell then goes on to give rise to 20 additional cells, includ-ing 9 neurons of the ring ganglion. The progeny of the Abarpa blastomere, like most of the progeny of the AB lineage, lie primarily on the surface of the embryo prior to 200 min of development. At this time, the cells on the ventral and lat-eral sides of the embryo move inside and become the nervous system, whereas the AB progeny that remain on the surface spread out to form the hypodermis, a syncytial covering of the animal. Most of the neurons arise in this way; of the 222 neu-rons in the newly hatched C. elegans, 214 arise from the AB lineage, whereas 6 are derived from the MS blastomere and 2 from the C blastomere.
DROSOPHILA
The development of the fruit fly, Drosophila, is character-istic of many arthropods. Unlike the embryos of the nema-tode, where cleavage of the cells occurs at the same time as nuclear divisions, the initial rounds of nuclear division in the Drosophila embryo are not accompanied by corresponding cell divisions. Instead, the nuclei remain in a syncytium up until just prior to gastrulation, three hours after fertilization. Prior to this time, the dividing nuclei lie in the interior of the egg, but they then move out toward the surface and a process known as cellularization occurs, and the nuclei are surrounded by plasma membranes. At this point the embryo is known as a cellular blastoderm.
The major part of the nervous system of Drosophila arises from cells in the ventrolateral part of the cellular blastoderm (Figure 1.4, top). Soon after cellularization, the ventral fur-row, which marks the beginning of gastrulation, begins to form ( Figure 1.4 , middle). At the ventral furrow, cells of the future mesoderm fold into the interior of the embryo. The process of invagination occurs over several hours, and the invaginat-ing cells will continue to divide and eventually will give rise to the mesodermal tissues of the animal. As the mesodermal cells invaginate into the embryo, the neurogenic region moves from the ventrolateral position to the most ventral region of the animal ( Figure 1.4 ). The closing of the ventral furrow creates the ventral midline, a future site of neurogenesis. On either side of the ventral midline is the neurogenic ectoderm, tissue that will give rise to the ventral nerve cord, otherwise known as the central nervous system (Figure 1.5 ). A continu-ation of the neurogenic region into the anterior of the embryo, is called the procephalic neurogenic region and gives rise to the cerebral ganglia or brain.
Drosophila neurogenesis then begins in the neurogenic region; some cells enlarge and begin to move from the out-side layer into the inside of the embryo ( Figure 1.5 ). At the beginning of neurogenesis, the neurogenic region is a sin-gle cell layer; the first morphological sign of neurogenesis is that a number of cells within the epithelium begin to increase in size. These larger cells then undergo a shape change and squeeze out of the epithelium. This process is called delami-nation and is shown in more detail in Figure 1.5 . The cells that delaminate are called neuroblasts and are the progenitors that will generate the nervous system. In the next phase of neurogenesis, each neuroblast divides to generate many prog-eny, known as ganglion mother cells (GMCs). Each GMC then generates a pair of neurons or glia. In this way, the entire central nervous system of the larval Drosophila is generated. However, the Drosophila nervous system is not finished in the larva, but rather additional neurogenesis occurs during metamorphosis. Sensory organs, like the eyes, are generated from small collections of cells in the larva (called imaginal discs) that undergo a tremendous amount of proliferation dur-ing metamorphosis to generate most of what we recognize as an adult fly.
VERTEBRATES
All vertebrate embryos develop in a fundamentally similar way, though at first appearance they seem to be quite differ-ent. In this section we will review the development of several different vertebrates: amphibians, fish, birds, and mammals. In all of these animals, multiple cleavage divisions generate a large number of cells from the fertilized oocyte. However, while gastrulation in all of these animals is basically con-served, the details of the cellular movements during this phase can look quite different.
Amphibian eggs are like those of many animals in that the egg has a distinct polarity with a nutrient-rich yolk concentrated at the “vegetal” hemisphere and a relatively yolk-free “animal” hemisphere. After fertilization, a series of rapid cell divisions, known as cleavages, divides the fertilized egg into blastomeres. The cleavage divisions proceed 3
DEVELOPMENT OF THE NERVOUS SYSTEM
Motorneuron, ventral nerve cord
Fig. 1.3 The next phase of development of the C. elegans worm also highlights the shared lineages of hypodermis and neurons. During gastrulation, the MS, E, C, and D blastomeres all migrate into the interior of the embryo, while the prog-eny of the AB blastomeres spread out over the external surface. Once the embryo starts to take form, sections through the embryo show the relationships of the cells. The neurons are primarily derived from the ventrolateral surface, through the divisions of the AB progeny cells. As these cells are generated, they migrate into the interior and form the nerve rings. A typical lineage is also shown. The Abarpa blastomeres undergo five rounds of division, to generate 9 neurons and 10 hypodermal cells. Neural lineages are shown in red.
1. NEURAL INDUCTION
Dorsal Cellular blastoderm D
(D) Ectoderm
Neurogenic
region
Anterior Posterior
(A) (P)
Mesoderm VVentral
(V) Gastrulation
Mesoderm
Neurogenic region Neurogenesis Epidermis
Gut
Adult
Mesoderm
NeurogenicNeuroblast regionBrain
Larva Gut
Ventral nerve cord
Fig. 1.4 The nervous system of the Drosophilais derived from the ventrolat-eral region of the ectoderm. The embryo is first (top) shown at the blastoderm stage, just prior to gastrulation. The region fated to give rise to the nervous system lies on the ventral-lateral surface of the embryo (red). The involution of the mesoderm at the ventral surface brings the neurogenic region closer to the midline. Scattered cells within this region of the ectoderm then enlarge, migrate into the interior of the embryo, and divide several more times to make neurons and glia. These neurons and glia then condense into the ganglia of the mature ventral nerve cord (or CNS) in the larva and the adult.
less rapidly through the vegetal hemisphere, and by the time the embryo reaches 128 cells, the cells in the animal half are much smaller than those of the vegetal half (Figure 1.6). The embryo is called a blastulaat this stage. The process by which the relatively simple blastula is transformed into the more complex, three-layered organization shared by most animals is called gastrulation.During this phase of development, cells on the surface of the embryo move actively into the center of the blastula. The point of initiation of gastrulation is identi-fied on the embryo as a small invagination of the otherwise smooth surface of the blastula, and this is called the blastopore (Figure 1.6). In amphibians the first cells to invaginate occur at the dorsal side of the blastopore (Figure 1.6), opposite to the point of sperm entry. The dorsal side of the blastopore has a special significance for the process of neural induction and much more will be said about this in this chapter.
The involuting cells lead a large number of cells that were originally on the surface of the embryo to the interior (Figure 1.6). The part of the blastula that will ultimately reside in the interior of the embryo is called the involuting
Dorsal
(D)
Anterior
(A)
Epidermis Ventral
(V)
Mesoderm Neurogenic region GMC1 GMC2
GMC1 GMC2 GMC3
Nb Nb
Nb Nb
Fig. 1.5
The neuroblasts of the Drosophilaseparate from the ectoderm by a process known as delamination. The neuroblasts enlarge relative to the surrounding cells and squeeze out of the epithelium. The process occurs in several waves; after the first set of neuroblasts has delaminated from the ecto-derm, a second set of cells in the ectoderm begins to enlarge and also delami-nates. The delaminating neuroblasts then go on to generate several neurons through a stereotypic pattern of asymmetric cell divisions. The first cell divi-sion of the neuroblast produces a daughter cell known as the ganglion mother cell, or GMC. The first ganglion mother cell divides to form neurons, while the neuroblast is dividing again to make another GMC. In the figure, the same neuroblast is labeled through its successive stages as Nb, while the GMCs are numbered successively as they arise.
marginal zone(IMZ). Most of these cells will give rise to mesodermally derived tissues, like muscle and bone. The first cells to involute crawl the farthest and produce the mesoderm of the anterior part of the animal (i.e., the head). The later involuting IMZ cells produce the mesoderm of more posterior regions, including the tail of the tadpole. At this point in development, the neural plate of the vertebrate embryo still largely resembles the rest of the surface ecto-derm. However, shortly after its formation, the neural plate begins to fold onto itself to form a tubelike structure, the 5
DEVELOPMENT OF THE NERVOUS SYSTEM
Blastula
Blastocoel
Animal
Ventral Dorsal VegetalVegetal
Animal
Endoderm
Neural plate
Yolk plug Ventral mesoderm
Blastopore
Neurogenic region
Blastocoel Sperm
Mesoderm
Involuting Marginal Zone (IMZ)
Archenteron
Mesoderm
Blastopore
Archenteron
Ectoderm
Fig. 1.6 The development of the central nervous system, brain, and spinal cord in a frog embryo is shown from the egg cell to the adult. After a series of cleavage divisions produce a blastula, a group of cells known as the involuting marginal zone, or IMZ, grow into the interior of the embryo at a point known as the blastopore. This process of gastrulation is shown in two cross sections. The involuting cells go on to form mesodermal tissues (blue) and induce the cells of the overlying ectoderm to develop into neural tissue, labeled as the neurogenic region (red). After the process of neural induction, the neurogenic region is known as the neural plate and is now restricted to giving rise to neural tissue. A cross section of the embryo at the neural plate stage shows the relationships between the tissues at this stage of development. The neural plate goes on to gen-
erate the neurons and glia in the adult brain and spinal cord.
neural tube (Figure 1.7). Much more will be said about the neural tube and its derivatives and shape changes in the next two chapters. For now, suffice it to say that this tube of cells gives rise to nearly all the neurons and glia of vertebrates. Another source of neurons and glia is the neural crest, a group of cells that arises at the junction between the tube and the ectoderm ( Figure 1.7 ). The neural crest is the source of most of the neurons and glia of the peripheral nervous sys-tem, whose cell bodies lie outside the brain and spinal cord. This tissue is unique to vertebrates and has the capacity to generate many diverse cell types; we will have more to say
6 about the neural crest in later chapters.
The complex tissue rearrangements that occur during gastrulation in the amphibian occur in other vertebrates in fundamentally the same way. However, the details of these movements can be quite different. Much of the difference in cell movements lies in differences in the amount of yolk in the egg. Fish and bird embryos have a substantial amount of yolk; since the cleavage divisions proceed more slowly through the yolk, these animals have many more cleavage divisions in the animal pole than in the vegetal pole. In zebrafish embryos, the blastomeres are situated at the top of the egg, and as devel-opment proceeds these cells divide and spread downward over the surface of the yolk cells; this downward spreading is called
|
|
|